A smorgasboard of major experiment results
Posted by David Zaslavsky on — Edited — CommentsWe’re now halfway into Quark Matter 2012, and many of the presentation slots (at least the ones I’ve looked at) have been devoted to the experimental groups presenting their new results. In heavy ion physics, the major groups are the STAR and PHENIX collaborations at RHIC, and ATLAS, CMS, and ALICE (which I’ve learned is pronounced “ah-LEES,” not “AL-iss”) at the LHC.
Naturally, the experts who are interested in these things will just go straight to the conference page and look at the presentations — heck, they’re pretty much all here in Washington anyway. So I’m going to try to explain some of these results in a way that makes them comprehensible for non-experts (although, apparently unlike some of my fellow conferencegoers, I’ll give you enough credit to assume you know what atoms are).
What Quark Matter is all about
The main focus of the Quark Matter conference is, of course, quarks, and also gluons: the most fundamental particles that make up atomic nuclei. How do they organize themselves into protons and neutrons and then into nuclei? How do the properties of those atomic nuclei emerge from their internal structure? And what really happens when you smash two nuclei together, anyway?
To answer all these questions, we need to examine situations in which quarks and gluons come together in large numbers. One way to do this is to collide hadrons (particles made of quarks and gluons) together at high speeds, with very large amounts of energy. Because of the quantum nature of hadrons, they appear to consist of more and more particles (roughly speaking) as you subject them to higher and higher energies. The other way is to start with large particles that naturally contain lots of quarks and gluons, namely entire atomic nuclei instead of just protons (as were used to discover the Higgs boson, for example). Or best of all, do both! That’s what relativistic heavy ion collisions are all about, and it is these collisions that underlie the majority of the results being presented at Quark Matter.
RHIC experiments
Of the five major experiments presenting results at Quark Matter, two of them, STAR and PHENIX, are located at the Relativistic Heavy Ion Collider facility. RHIC has collided a variety of different kinds of particles in the past, most notably gold nuclei with other gold nuclei (Au-Au), and with deuterons (d-Au), as well as some “ordinary” proton-proton (p-p) collision. More recently, they’ve also collided uranium on uranium (U-U) and copper on both copper (Cu-Cu) and gold (Cu-Au), and the results of these collisions are among the new ones presented at this conference.
LHC experiments
There are three experiments doing heavy ion physics at the Large Hadron Collider, which has spent about a month out of each of the past two years (2010 and 2011) colliding lead ions (Pb-Pb) instead of protons. The two general-purpose experiments, ATLAS and CMS (both of which made the announcements of the Higgs boson discovery), also collect data during these heavy ion runs, and there is also ALICE, a specialized experiment for heavy ion physics. Representatives of all three are presenting the analyses of the 2011 data for the first time during Quark Matter 2012.
Results
With the general goal of understanding the structure of protons, neutrons, and nuclei, there are a variety of specialized measurements that heavy ion physicists make using the data these experiments collect. Here’s a sample, just what I can put together quickly.
Nuclear modification factors
We already have a basic idea of how to characterize the behavior of protons in particle collisions, since the LHC and before that the Tevatron and other accelerators have been working with them for many years. So it makes sense to try to understand nuclei by relating their behavior to that of protons. One of the quantities commonly calculated to do this is \(R_{AA}\), the nuclear modification factor, which represents how often a particular sort of particle is produced in a nuclear collision relative to how often it’s produced in proton-proton collisions.
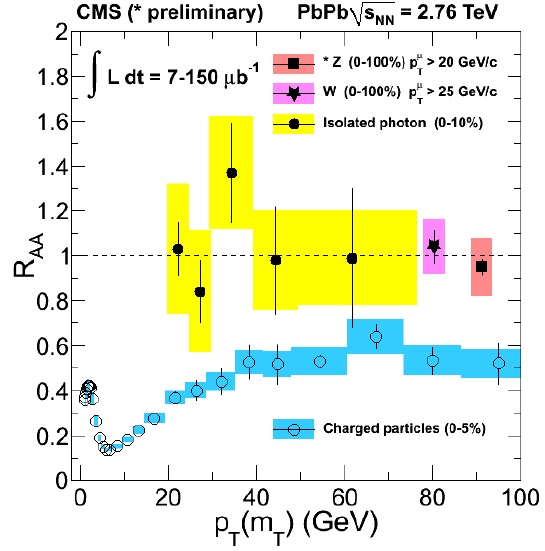
This image shows \(R_{AA}\) for various sorts of particles (identified by different colors) as measured by the CMS experiment. It shows that high-momentum charged particles are emitted about half as often as you’d naively expect based on a model of a bunch of individual proton collisions, and that this ratio is fairly constant over momentum for a significant range.
Jet quenching
In every collision, we examine the structure of the particles that go in by looking at the particles that come out. But in heavy ion collisions specifically, we want to look at the particles that come out from the center of the collision, passing through the rest of the nuclei on the way, since they encode information about the internal structure of the nuclei as they pass through it. These particles tend to be clustered into jets.
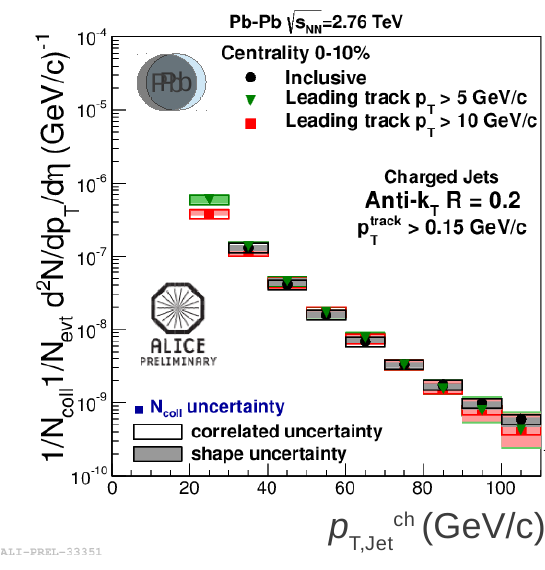
Here we see a measurement of the most energetic jets from the ALICE experiment. The different colors indicate different filters on the momentum of the jet. Use of the LHC allows these measurements to be taken at higher energies (so, more points on the graph) than was previously possible.
Quark-gluon plasma
One of the major goals of relativistic heavy ion physics is studying the evolution of the quark-gluon plasma. Quark-gluon plasma — which, despite the name, is not a plasma, it actually acts more like a very stiff liquid — is an exotic state of matter that occurs immediately after the collision of two heavy ions. The study of the QGP falls under the general area of determining the details of the QCD phase diagram, which is a summary of the various states of matter that quarks and gluons can form at high temperatures and densities.
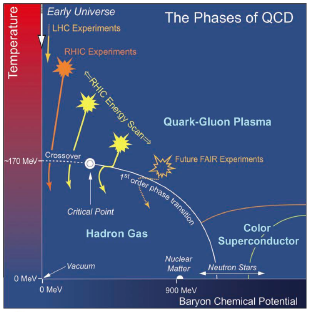
The quark-gluon plasma specifically is the focus of a lot of attention because the same state of matter would have filled the universe a fraction of a second after the Big Bang, and so understanding how the quark-gluon plasma behaves in heavy ion collisions tells us something about how our universe got to be the way it is today.
Physicists are interested in determining the QGP’s viscosity and other transport properties that affect how it flows, as well as understanding how it forms, how its shape and energy distribution changes as it evolves, how and how fast it comes to thermal equilibrium, and how it dissipates at the “end” of the collision. That is accomplished by studying the jets emerging from the collision, not only with respect to jet quenching (since the manner in which jets are dissipated tells you about the properties of the medium doing the dissipating), but also by looking at the angular distribution of these jets. You can analyze certain modes from the jet distribution, the angular harmonics shown in the picture at the right, which can be related to the structure of the quark-gluon plasma.
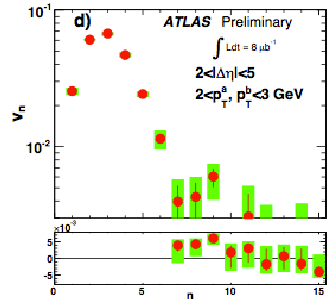
This picture (apologies for the low quality, I’ll see if I can fix that) shows the flow coefficients \(v_n\) as a function of \(n\). Higher numbered coefficients are modes with more bumps. You can see from the graph that the dominant modes in this data are \(v_2\), \(v_3\), and \(v_4\), and I will add that \(v_2\) is of particular interest because it measures the elliptic flow, which includes how strongly the jets are directed along, say, the vertical axis as opposed to the horizontal axis (or any other set of perpendicular directions). This relates to the compression of the QGP, because a direction along which a lot of high-energy jets emerge is a direction in which the QGP would have been highly compressed.
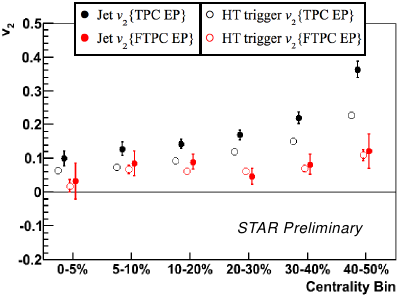
This next picture shows that elliptic flow coefficient \(v_2\) as a function of centrality, which indicates how well the colliding nuclei are lined up with each other (a high centrality percentage means they hit head-on, low centrality means they strike a glancing blow). At high centrality, you can see that there is a strong bias toward one direction as opposed to its perpendicular direction.
Saturation physics
Finally, there is my research area, saturation physics, which is all about determining the contents of a proton or nucleus under conditions where it is tightly packed with particles, so to speak. This is a relatively minor contribution to heavy ion physics, but still important, because the phenomenon of saturation (how proton/nuclear structure changes under these dense conditions) is a key part of understanding why protons, neutrons, and nuclei have the properties they do. Most of this research involves looking for particular signatures of saturation. For example, the graphs below show a prediction for \(R_{\mathrm{pPb}\) (the \(R_{AA}\) I explained above, for proton-lead collisions) demonstrating how we might see a particular effect of saturation. The red line is a prediction based on a pure proton model, basically what would happen if the nucleus consisted of separate noninteracting protons, and the other lines are various more complex predictions that take into account saturation effects coming from having so many protons and neutrons so close together.
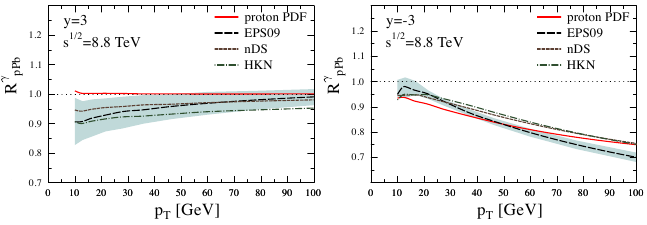
And much more…
Naturally, what I’ve presented above is only a tiny extract from just a few of the many detailed experimental results being presented at Quark Matter. All the presentations are, or will soon be, available from the conference web page, but given the highly specific nature of this research, it’s probably hard to appreciate their significance if you’re not a specialist in the field (and sometimes even if you are). Hopefully I’ve been able to change that just a little bit.